Geotechnical Engineering
Geotechnical engineering is a critical branch of civil engineering that deals with the behavior and properties of earth materials, such as soil and rock. It plays a crucial role in the design, construction, and maintenance of infrastructure, ensuring that projects are built on a solid foundation that can support the intended loads and resist the stresses imposed by environmental conditions. Geotechnical engineers assess and manage the risks associated with soil properties, slope stability, and potential hazards such as landslides, earthquakes, and subsidence. Their assessment helps in selecting appropriate materials, design methods, and construction techniques that contribute to the safety, longevity, and cost-effectiveness of structures.
The importance of geotechnical engineering extends beyond the safety and performance of structures, as it is also crucial in the preservation of our natural resources and the protection of the environment. Geotechnical engineers work closely with environmental engineers to develop effective strategies for managing soil and groundwater contamination, waste disposal, and land reclamation. They are also involved in the design and implementation of erosion control measures, flood protection systems, and sustainable construction practices that minimize environmental impacts and promote conservation of natural resources.
Moreover, geotechnical engineering plays a pivotal role in major public works and urban development projects, addressing complex challenges associated with rapid urbanization, climate change, and population growth. By providing valuable expertise in the evaluation of soil conditions and the design of suitable infrastructure, geotechnical engineers help to ensure that our cities and communities can thrive and adapt to changing conditions while minimizing the risks posed by natural hazards and man-made challenges. Their work contributes to the development of resilient and sustainable built environments that support the social, economic, and environmental needs of our society.
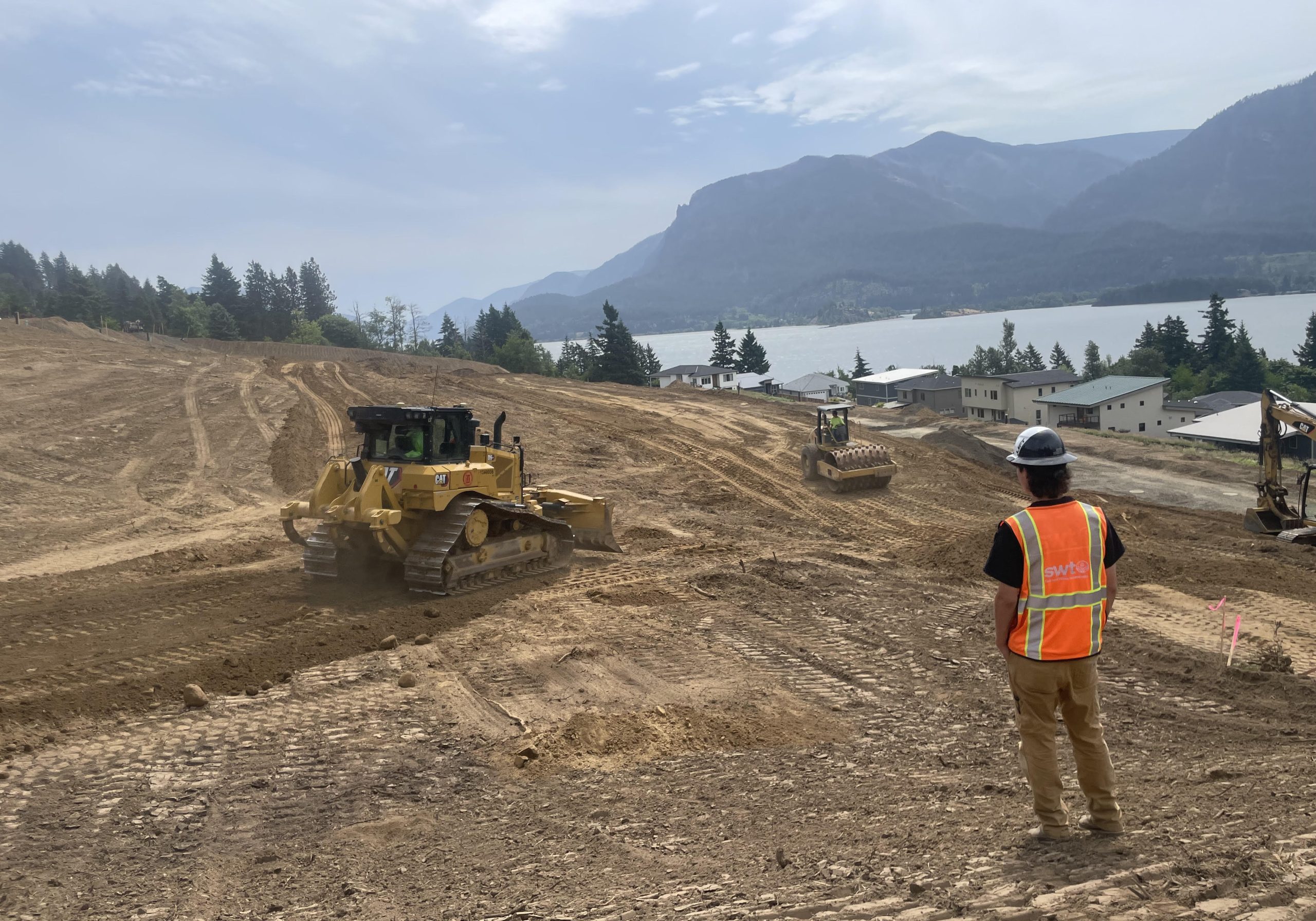
Soil science and its role in geotechnical engineering
Soil science is a multidisciplinary field that investigates the physical, chemical, and biological properties of soils and their relationships with the environment. It is an essential component of geotechnical engineering, as a thorough understanding of soil properties is fundamental to the analysis and design of infrastructure. Soil properties, such as texture, structure, permeability, and compressibility, determine how the soil will behave under various loading conditions and the extent to which it can support structures.
Soil scientists and geotechnical engineers often collaborate to study the characteristics of different soil types, evaluate their suitability for construction, and develop strategies for mitigating risks associated with problematic soils. For example, expansive soils, which swell and shrink with changes in moisture content, can cause severe damage to structures built on or within them. A soil scientist can help identify these soils and provide recommendations for appropriate construction techniques or ground improvement methods to minimize potential issues.
Furthermore, soil science plays a vital role in the development of sustainable and environmentally-friendly construction practices. The proper understanding and management of soils can support the implementation of low-impact development strategies, such as green roofs, permeable pavements, and bioretention systems, which help to conserve natural resources, mitigate pollution, and promote biodiversity. Soil science also contributes to the assessment of potential environmental impacts and remediation strategies for contaminated sites, ensuring that our environment remains healthy and vibrant for future generations.
Differentiating between geotechnical engineering and soil science
While geotechnical engineering and soil science share a focus on the study and understanding of soils, they can be distinguished by their specific objectives and areas of expertise. Geotechnical engineering is a specialized discipline within civil engineering that primarily deals with the design and construction of infrastructure, such as buildings, roads, bridges, and dams. It involves the application of principles from soil mechanics, rock mechanics, and engineering geology to analyze and predict the behavior of earth materials under various loading and environmental conditions.
In contrast, soil science is a broader, interdisciplinary field that encompasses the study of soils in relation to their physical, chemical, and biological properties, as well as their interactions with plants, animals, and microorganisms. Soil scientists investigate a wide range of topics, from the classification and mapping of soil types to the impact of agricultural practices on soil fertility, carbon sequestration, and nutrient cycling. While soil scientists may be involved in the assessment of soil conditions for engineering purposes, their scope of work extends beyond the realm of civil engineering and is also relevant to fields such as agriculture, environmental management, and earth system sciences.
Despite these differences, the expertise of both geotechnical engineers and soil scientists is crucial for creating safe, sustainable, and resilient built environments. By collaborating and integrating their respective knowledge, they can develop innovative solutions that address the complex challenges associated with soil behavior, site conditions, and geotechnical risk management. Ultimately, the synergy between geotechnical engineering and soil science contributes to the development of a more holistic understanding of our natural and built environments and promotes a greater appreciation for the importance of soils in our everyday lives.
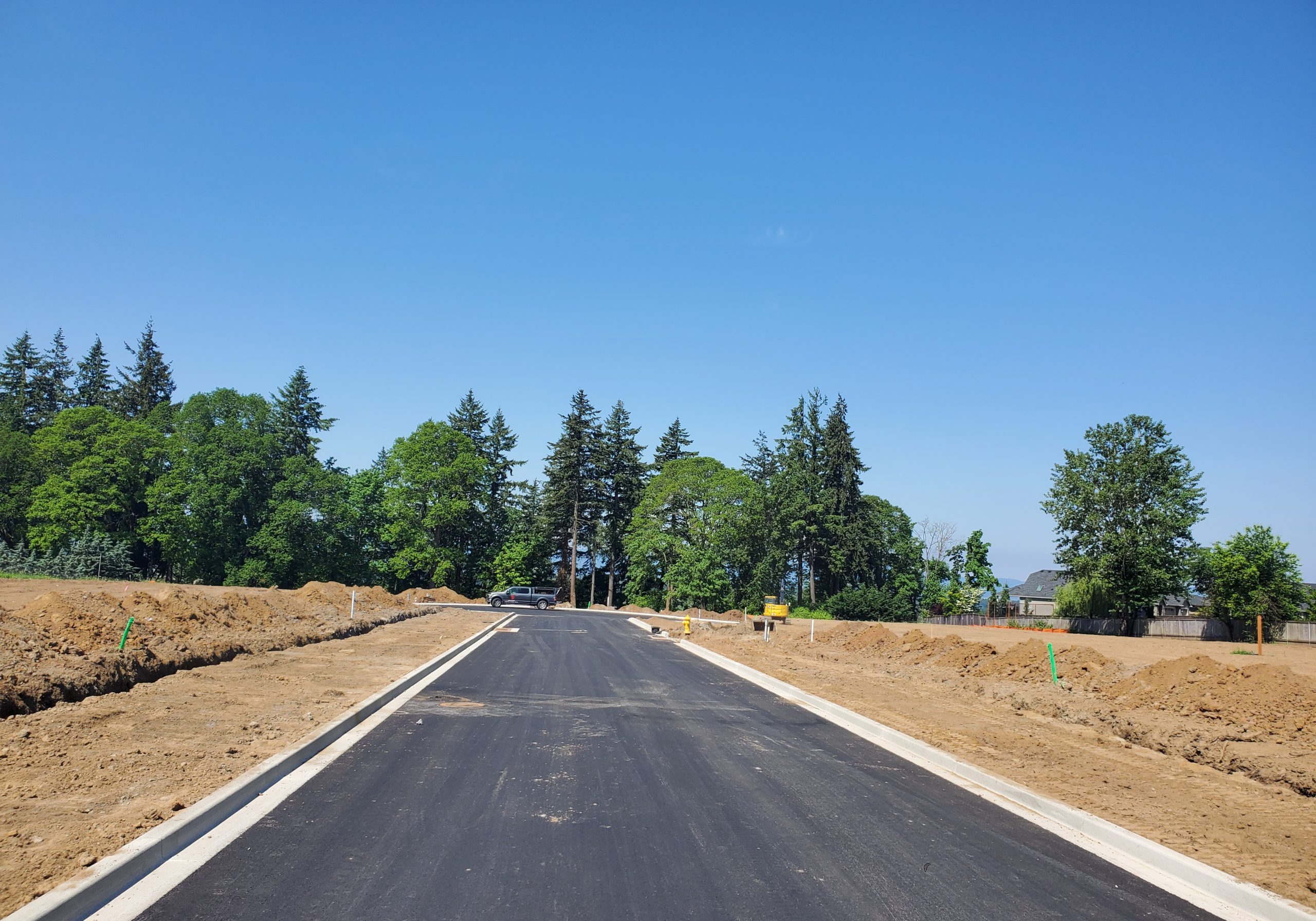
Key elements and principles of geotechnical engineering
Geotechnical engineering encompasses several key elements and principles that guide the analysis, design, and construction of infrastructure. One fundamental principle is the concept of bearing capacity, which refers to the ability of a soil or rock to support the weight of a structure without experiencing excessive deformation or failure. Geotechnical engineers must evaluate bearing capacity to determine the most suitable foundation type and size, as well as potential ground improvement methods that may be required to enhance soil strength and stability.
Another essential aspect of geotechnical engineering is the assessment and management of slope stability. Slope stability analysis involves evaluating the stability of natural and man-made slopes, identifying potential failure mechanisms, and designing appropriate mitigation measures, such as retaining walls, drainage systems, or slope reinforcement. This analysis is critical for ensuring the safety of structures and infrastructure located on or adjacent to slopes, as well as for protecting the surrounding environment and communities from the risks associated with landslides and erosion.
Lastly, geotechnical engineers must consider the effects of environmental factors, such as groundwater and climate, on the performance of structures and earthworks. Groundwater fluctuations can influence soil strength, settlement, and drainage, while climate conditions, such as freeze-thaw cycles and precipitation, can lead to changes in soil moisture content, erosion, and slope instability. By understanding these interactions and incorporating appropriate design considerations, geotechnical engineers can develop infrastructure solutions that are resilient and adaptable to the changing conditions of our environment.
Soil Properties and Classification
Soil composition
Soil is a complex and dynamic natural material composed of an intricate mix of minerals, organic matter, water, and air, each playing a critical role in the soil's overall behavior and engineering properties. The mineral component primarily consists of weathered rock fragments, clay minerals, and quartz, which account for the soil's mechanical properties, such as strength, compressibility, and permeability. Organic matter, including decaying plant and animal remains, contributes to the soil's fertility and overall nutrient content.
The presence of water in soil regulates its consistency, affecting its behavior under various loading conditions. Water fills the voids between soil particles, creating a saturated or unsaturated environment depending on the water content. When the soil is saturated, it becomes weaker and more susceptible to deformation under loading, thus requiring careful consideration in geotechnical engineering applications. Alternatively, air-filled voids in the soil influence its thermal, electrical, and acoustic characteristics, allowing for the exchange of gases required for various biological processes and improving workability in construction projects.
The balance between the four main components of soil – minerals, organic matter, water, and air – play a significant role in determining the soil's characteristics and engineering performance. Understanding the composition of a specific soil sample can help geotechnical engineers and soil scientists predict its behavior under different conditions, allowing for appropriate design and construction practices. Proper assessment of soil composition is essential for successful geotechnical engineering projects such as foundation design, earth retaining structures, and slope stability analysis.
Soil types and their engineering properties
There are numerous soil types, each with distinct engineering properties that influence their suitability for different geotechnical applications. The three most common soil types are sands, silts, and clays, classified based on their particle size, plasticity, and mineralogy. Sands consist of coarse-grained particles that exhibit high permeability, low compressibility, and substantial strength when compacted, making them suitable for foundations and as backfill for retaining walls.
Silts, composed of fine-grained particles, pose unique challenges in geotechnical engineering due to their intermediate properties between sands and clays. Although they exhibit higher compressibility and lower permeability than sands, they still have sufficient strength when compacted correctly. Clay soils, composed of even smaller particles and exhibiting plastic behavior, possess high compressibility, low permeability, and are highly sensitive to changes in water content, making them prone to swelling and shrinking with fluctuations in moisture conditions. Clays are typically challenging to work with in construction, requiring specific ground improvement techniques and unique design considerations.
In addition to the primary soil types mentioned above, engineers may also encounter gravels, peat, and expansive soils, each presenting its unique set of engineering properties and challenges. Understanding the different soil types and their respective engineering properties enables geotechnical engineers to properly design and implement construction projects, ensuring the stability and longevity of structures built on these soils.
Soil classification systems
Soil classification systems provide a standardized method for categorizing soils based on their physical and engineering properties, allowing for more effective communication among geotechnical engineers and soil scientists. Several classification systems exist, each with its unique set of parameters, including grain size distribution, plasticity, and mineral content. Two widely recognized systems are the Unified Soil Classification System (USCS) and the American Association of State Highway and Transportation Officials (AASHTO) system.
The USCS, developed by the U.S. Army Corps of Engineers and the U.S. Bureau of Reclamation, classifies soils into 15 primary groups based on their particle size distribution and plasticity. Each soil type is designated by one or two letters (e.g., GW, CL) representing the specific categories of grain size (G, S, M, or C) and plasticity (W, L, H, or O). This system is widely used for geotechnical engineering purposes, providing engineers with valuable information regarding soil behavior and its suitability for various construction projects.
The AASHTO classification system, designed specifically for use in highway and transportation-related construction, focuses on the soil's performance properties, such as strength, shrink-swell potential, and frost susceptibility. This system classifies soils into seven groups (A-1 to A-7) based on particle size distribution and plasticity index, with additional subgroups to further specify soil characteristics. Choosing the appropriate classification system for a specific project depends on the desired application and engineering requirements, with the common goal of enabling effective communication and decision-making in geotechnical engineering and soil science.
Importance of soil properties to geotechnical engineering
A clear understanding of soil properties is fundamental to the success of geotechnical engineering projects, as the behavior of soil directly influences the stability and performance of structures built upon it. Soil properties, such as strength, compressibility, permeability, and plasticity, determine the appropriate design and construction techniques for foundations, retaining walls, embankments, and other earth-related structures.
For instance, soil strength is vital for evaluating the bearing capacity of a foundation, ensuring that the structure's weight is adequately supported without excessive settlement. Similarly, soil compressibility and consolidation properties influence the settlement of structures and the time required for consolidation to occur, directly impacting the construction timeline and the overall structural integrity. Permeability and drainage characteristics of soil play a critical role in water flow and seepage, affecting slope stability, groundwater management, and the performance of retaining structures.
In summary, the various soil properties must be accurately assessed and considered during the design and construction phases of any geotechnical engineering project. Through comprehensive site investigations, soil sampling, and laboratory testing, engineers can attain a robust understanding of soil conditions, allowing for the application of appropriate design principles and construction techniques, ultimately ensuring the safety and longevity of the built environment.
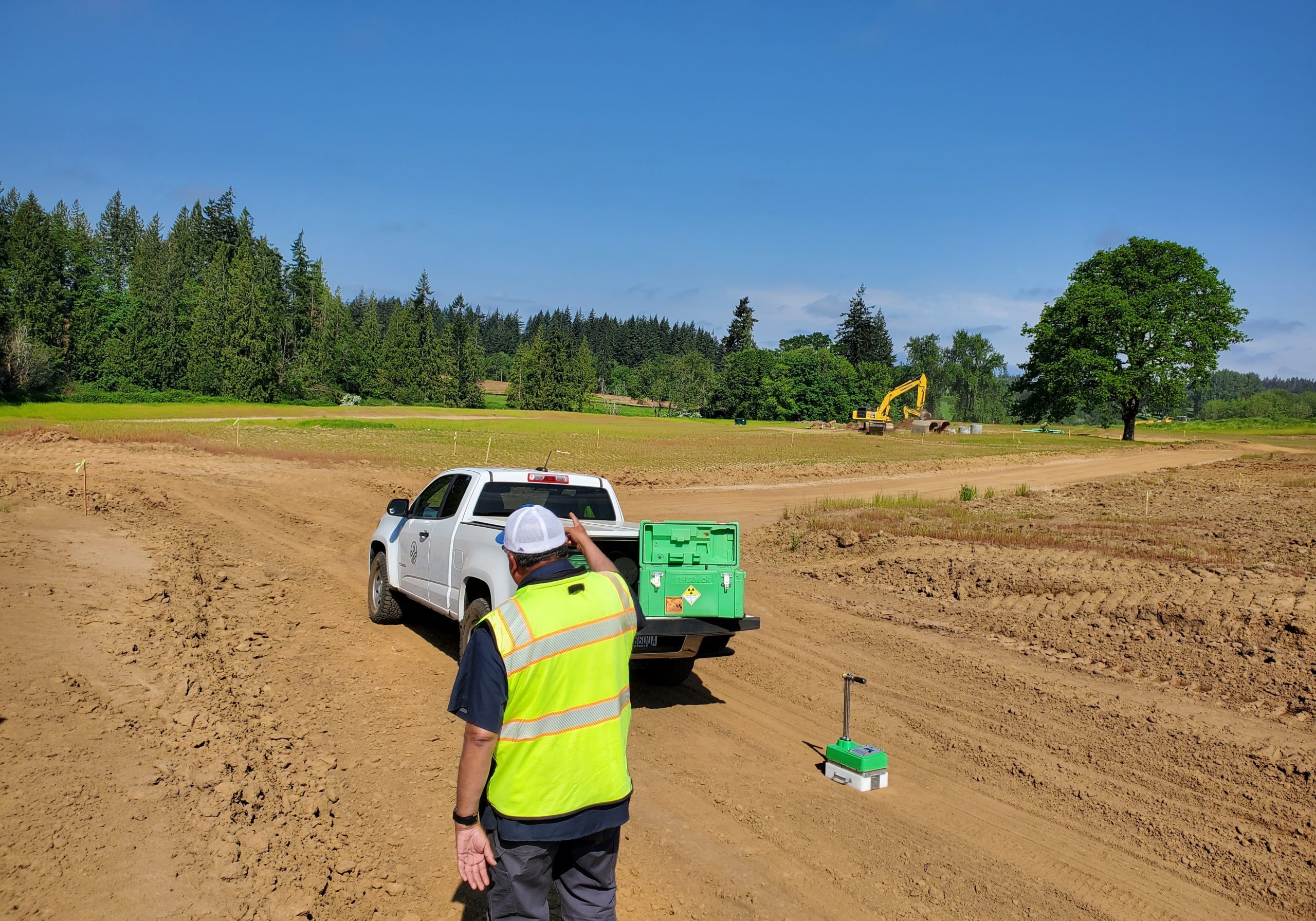
Site Investigation and Soil Sampling Techniques
Stages of a site investigation
A thorough site investigation is critical to the success of any geotechnical engineering and soil science project. When conducting a site investigation, there are several key stages to ensure accurate and comprehensive data collection. The first stage involves a preliminary investigation, which includes a review of available geological and topographic maps, historical data, and previous studies for the project area. This stage helps identify potential soil-related issues and hazards that may impact the project.
The second stage of a site investigation is the field investigation, where engineers and scientists visit the site to collect soil samples, conduct in-situ testing, and gather information about soil properties, groundwater conditions, and geologic features. In addition to sampling and testing, this stage may also involve the use of geophysical methods, such as seismic reflection or electrical resistivity, to provide more detailed information about subsurface conditions.
The final stage of a site investigation involves compiling and analyzing the data collected during the previous stages, as well as comparing the results with relevant geotechnical standards and guidelines. This stage allows engineers and scientists to develop a comprehensive understanding of the site's subsurface conditions and formulate appropriate design recommendations based on the findings. Ultimately, the goal of a site investigation is to provide a solid foundation for decision-making and risk assessment in geotechnical engineering and soil science projects.
Soil sampling methods
Numerous soil sampling methods are available, each suited to specific project requirements and soil conditions. One of the most common techniques is hand sampling, which involves using handheld tools, such as shovels, augers or soil probes, to collect samples from shallow depths. Hand sampling is ideal for preliminary site investigations, as it is relatively fast and low-cost but may not provide adequate information for more complex projects or deeper soil layers.
For larger projects or deeper sampling depths, drill rig methods, such as rotary drilling or direct push technology, are commonly used. Rotary drilling employs a rotating drill bit to cut through the soil, while direct push technology uses hydraulic force to advance a sampler into the ground, collecting soil cores as it goes. These methods provide more representative samples and can penetrate denser, harder soils or bedrock. Additionally, cone penetration testing (CPT) is a popular in-situ testing method for evaluating soil properties, such as strength and compressibility, by continuously pushing a cone-tipped probe into the ground while monitoring the resulting resistance.
In some cases, specialized sampling methods may be required to address unique site conditions, environmental concerns or regulatory requirements. For example, undisturbed sampling, which aims to preserve the natural structure and properties of the soil, may be necessary for evaluating sensitive soils or assessing slope stability. Environmental soil sampling methods, such as low-flow purging or passive samplers, can be employed to collect samples for contaminant analysis, while considering potential impacts on groundwater resources and ecosystems. Ultimately, the choice of soil sampling method will depend on the project goals, site conditions, and budget constraints.
Laboratory testing of soil samples
Once soil samples have been collected, laboratory testing plays a vital role in characterizing their properties and informing geotechnical engineering design. A wide variety of laboratory tests can be performed, depending on the project's specific needs and requirements. Common tests include grain size analysis, which determines the distribution of particle sizes in a soil sample; Atterberg limits, which measure the consistency of fine-grained soils at various water contents; and compaction tests, which evaluate the soil's ability to undergo densification under applied loads.
Other laboratory tests focus on evaluating the strength and deformation properties of soils. Examples include unconfined compression tests, which measure the compressive strength of cohesive soils; direct shear tests, which quantify the shear strength of soils along a pre-defined failure plane; and oedometer tests, which assess the soil's compressibility and consolidation properties. In addition to these mechanical tests, specialized tests may be conducted to assess the soil's chemical, biological, or environmental properties, such as contaminant concentrations, nutrient levels, or microbial activity.
It's important to note that laboratory testing should be conducted in accordance with established protocols and standards, such as those published by the American Society for Testing and Materials (ASTM) or the International Organization for Standardization (ISO). These standardized procedures help ensure the quality, reliability, and comparability of test results, which are critical for making informed decisions in geotechnical engineering and soil science projects.
Interpreting and analyzing soil investigation results
Interpreting and analyzing soil investigation results is a crucial step in developing an accurate understanding of subsurface conditions and informing geotechnical design decisions. This process typically involves compiling and evaluating the data from field and laboratory tests, as well as comparing the findings against relevant geotechnical standards, guidelines, and project requirements. Statistical methods and software tools can be employed to facilitate data analysis, visualize trends in the results, and identify potential correlations or anomalies.
Once the data has been analyzed, it is essential to develop a clear and comprehensive geotechnical model that captures the site's subsurface conditions, soil layering, and key geotechnical properties. This model may be used to support the design of foundations, earth retaining structures, and other geotechnical elements, as well as to assess the suitability of the site for the proposed project. It is important to consider the inherent uncertainties and limitations associated with soil investigation data, such as sampling or testing errors and spatial variability, when interpreting the results and making design decisions.
Collaboration and communication are also critical throughout the data interpretation and analysis process. Geotechnical engineers and soil scientists should work closely with project stakeholders, including structural engineers, environmental specialists, and construction professionals, to ensure that the geotechnical model aligns with the overall project objectives and constraints. By integrating soil investigation results with other relevant information, such as geophysical or environmental data, project teams can develop a comprehensive understanding of site conditions, make informed design decisions, and manage geotechnical risks more effectively.
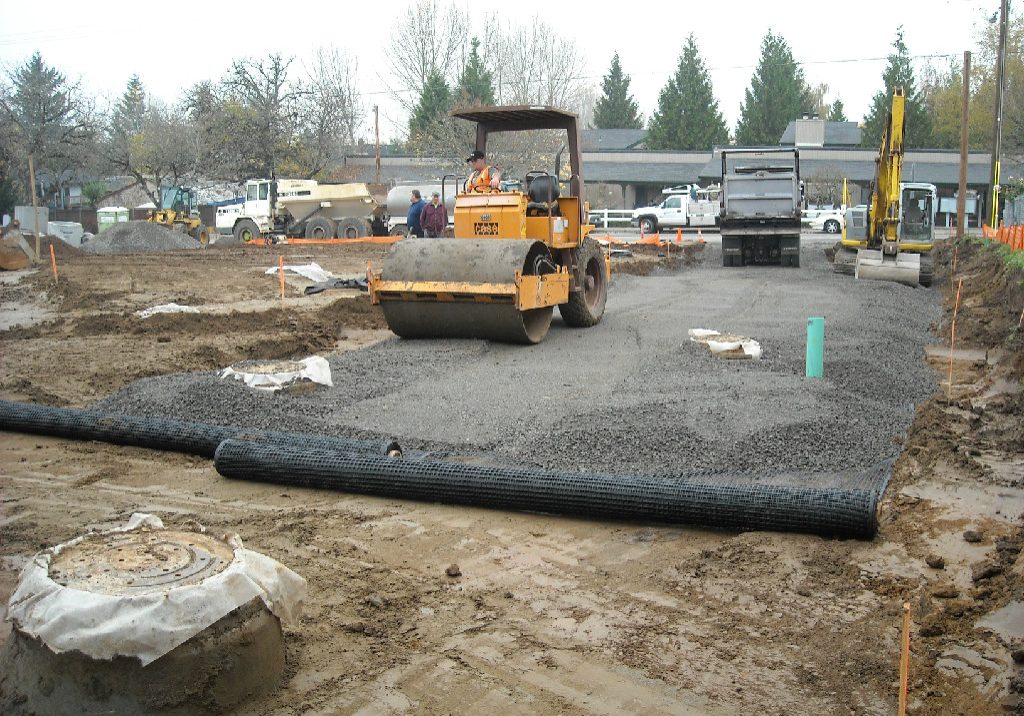
Foundations and Ground Improvement Techniques
Types and selection of foundations
In geotechnical engineering and soil science, the selection of an appropriate foundation type plays a critical role in ensuring the stability and longevity of a structure. The chosen foundation must be capable of bearing the load of the structure while taking into consideration the properties of the underlying soil. There are two main categories of foundations: shallow foundations and deep foundations. Shallow foundations, which include strip footing, isolated footing, and mat (raft) foundations, are used when the bearing capacity of the soil is sufficient for supporting the structure's loads at relatively shallow depths. They are typically suitable for lighter structures or when the soil has good bearing capacity near the surface.
On the other hand, deep foundations are employed when the near-surface soil has inadequate bearing capacity, or when the size and weight of the structure necessitate the load to be transferred to deeper, more stable soil layers or rock. Deep foundations include piles, drilled shafts, and caissons. Piles are long, slender structural members that transfer loads from the structure to the strong layer of soil or rock beneath. Drilled shafts, also known as bored piles or caissons, are large diameter, reinforced concrete elements that can carry heavy loads and resist lateral forces from wind, seismic events, or other dynamic loads.
When selecting a foundation type, engineers must consider several factors such as soil composition, bearing capacity, water table level, depth of the strong soil layer, and the type and magnitude of loads imposed by the structure. Thorough site investigation and soil analysis are critical components in determining the most suitable foundation type for a particular project. It's essential to choose a foundation type that provides adequate support, minimizes settlement, and ensures the overall stability of the structure under various conditions.
Ground improvement methods
Ground improvement methods are techniques employed to enhance the properties of the soil, making it more suitable for supporting structures and infrastructure. These methods aim to increase the strength, reduce settlement, and improve the overall performance of the soil. There are various ground improvement methods used in geotechnical engineering and soil science, which can be broadly classified into three categories: densification, reinforcement, and stabilization.
Densification involves compacting the soil, thereby increasing its density, strength, and stiffness. Techniques used for soil densification include vibratory methods (vibrocompaction), impact methods (dynamic compaction), and static methods (preloading or surcharging). Vibration and impact methods compact the soil by inducing vibrations, which rearrange the soil particles into a denser configuration. Surcharging involves placing additional weight on the ground to consolidate the soil and reduce settlement over time.
Reinforcement methods enhance the soil's strength by including external elements such as geosynthetics (geogrids, geotextiles) or natural materials (soil nails, rock bolts). These elements provide tensile strength, allowing for the stabilization of slopes, retaining walls, and other earth structures. Stabilization techniques involve the use of chemical additives, such as lime, cement, or fly ash, to increase the soil's strength and stiffness, making it more suitable for construction purposes. The choice of ground improvement method depends on the project requirements, soil characteristics, and environmental considerations.
Design of shallow and deep foundations
The design process of shallow and deep foundations is a critical aspect of geotechnical engineering and soil science as it ensures the long-term stability and performance of structures. The primary objective is to provide adequate support for the applied loads while minimizing differential settlement and ensuring the overall stability of the foundation system. The design process involves several steps, including site investigation, soil classification, bearing capacity evaluation, and foundation sizing.
For shallow foundations, the design process begins with determining the soil's bearing capacity, considering factors such as soil type, soil strength, and groundwater level. The required width, length, and depth of the foundation are then determined based on the structure's load and the soil's bearing capacity. In some cases, shallow foundations may require ground improvement techniques to enhance the soil's properties and ensure the stability of the structure.
Deep foundation design involves determining the type, size, and spacing of the foundation elements (piles, drilled shafts) based on the structure's load, the depth of the strong soil layer, and the soil properties. Load tests are often conducted to ensure the foundation elements can support the anticipated loads without excessive settlement or displacement. Additionally, lateral load resistance, buckling, and group effects for multiple foundation elements must be considered in the design process.
Monitoring and evaluation of foundation performance
Proper monitoring and evaluation of foundation performance are essential aspects of geotechnical engineering and soil science. They provide valuable insights into the actual behavior of the foundation under the applied loads, allowing engineers to validate design assumptions, detect issues, and implement appropriate mitigation measures if necessary. Foundation performance monitoring can be conducted during the construction phase, post-construction, or during the structure's lifetime.
Monitoring methods include measuring settlement, deflection, tilting, and strain within the foundation elements. These measurements can be obtained through various techniques such as surveying, inclinometers, strain gauges, and load cells. Additionally, non-destructive testing methods such as ground-penetrating radar, ultrasonic testing, and thermal imaging can be used to detect defects or changes in soil properties around the foundation.
Evaluation of foundation performance involves comparing the measured data to the expected behavior from the design stage, as well as the established tolerances for allowable settlement and deformation. If the monitoring results indicate performance issues or deviations from the design assumptions, appropriate corrective actions must be taken. These may include reinforcing the foundation, implementing ground improvement techniques, or in extreme cases, complete foundation replacement. Continuous monitoring and evaluation ensure foundation safety, stability, and optimal performance throughout the structure's lifetime.
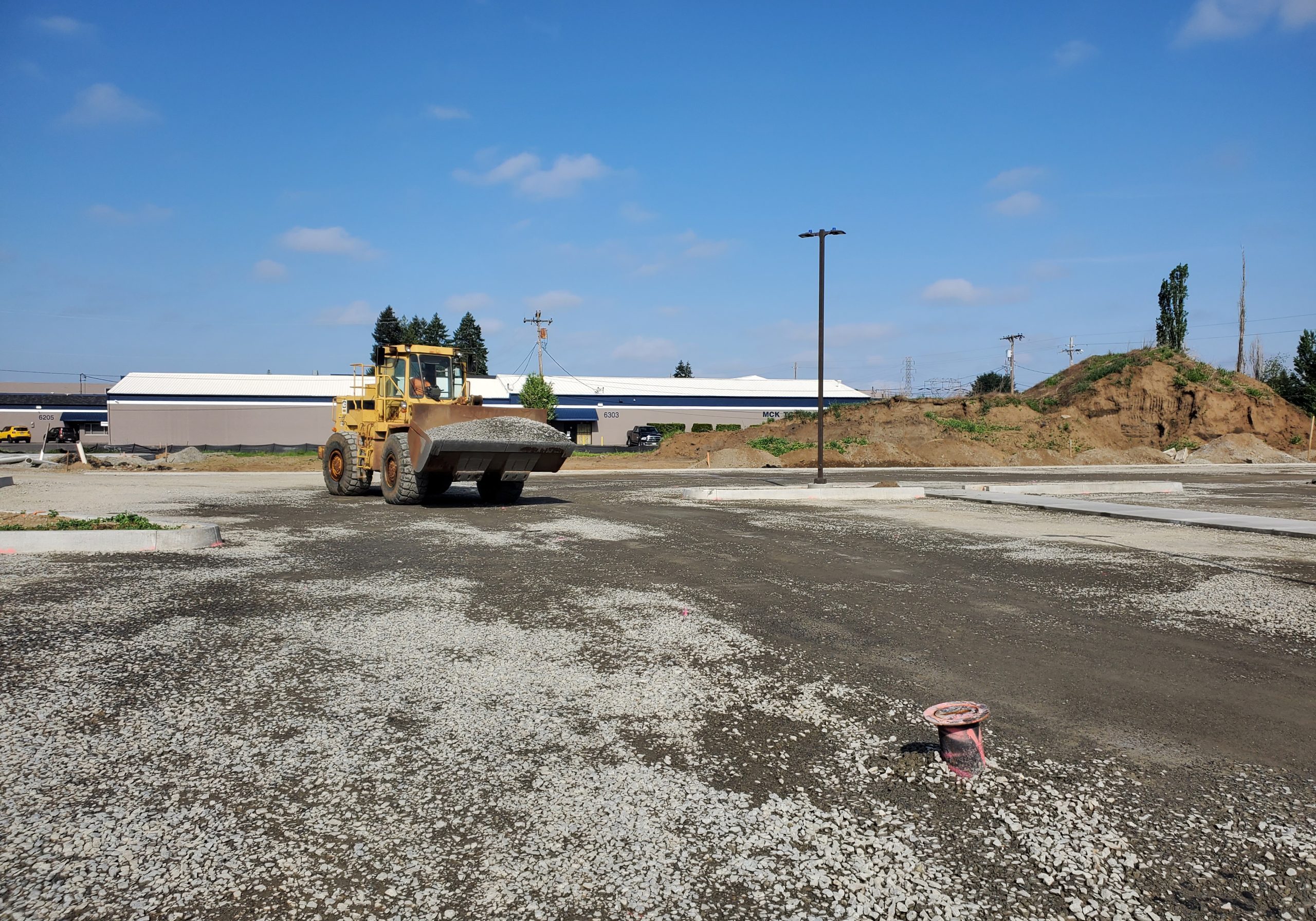
Slope Stability and Earth Retaining Structures
Causes and types of slope failure
Slope failure is a significant concern in geotechnical engineering and soil science as it can result in catastrophic consequences for infrastructure and human life. There are several causes for slope failures, including natural factors such as slope angle, soil composition, weathering, and groundwater conditions. Human-induced factors, such as excavation, loading, and changes in land use, can also contribute to slope instability. In addition, slope failures can be triggered by external events such as earthquakes, heavy rainfall, and freeze-thaw cycles.
There are various types of slope failures, each with its unique characteristics and failure mechanisms. One common type is rotational slumps, where a large mass of soil rotates and moves downslope due to a failure along a curved surface. Another type is translational slides, which occur when a soil mass moves along a flat or planar surface. Earthflows, debris flows, and mudflows are characterized by a downslope movement of soil and debris under the influence of gravity and water. Lastly, rockfalls and topples involve the sudden release and movement of rock fragments and boulders.
Understanding the causes and types of slope failure is crucial in geotechnical engineering and soil science. This knowledge allows engineers and scientists to accurately assess slope stability, design appropriate mitigation measures, and predict the likelihood of future failures. By thoroughly analyzing the factors that contribute to slope failure, professionals in these fields can ensure the safety and longevity of structures built on or near slopes, reduce the risk of property damage, and protect human life.
Slope stability analysis
Slope stability analysis is a critical component of geotechnical engineering and soil science, as it helps determine the safety and sustainability of a slope. The process typically involves understanding the soil mechanics, force balance, and potential failure mechanisms at play in a slope. Various analytical methods, both deterministic and probabilistic, are employed to assess slope stability, including limit equilibrium analysis, finite-element analysis, and probabilistic approaches.
Limit equilibrium analysis is a widely used deterministic method that involves dividing the slope into a series of slices, then calculating the factor of safety, which represents the ratio between the available shear strength and the shear stress acting on the potential failure surface. A factor of safety greater than 1 indicates a stable slope, while a factor of safety less than 1 signifies instability. Finite-element analysis, on the other hand, considers the mechanical behavior of soil and rock when subjected to loads and provides a more detailed understanding of the slope's response.
Probabilistic approaches to slope stability analysis account for uncertainties in soil properties, loading conditions, and other factors, providing a more comprehensive understanding of the potential risks and failure mechanisms. These methods are particularly useful in situations where data scarcity and variability pose significant challenges to using deterministic approaches. By employing a combination of analytical methods, geotechnical engineers and soil scientists can effectively assess slope stability, identify potential hazards, and design appropriate solutions for preventing slope failure.
Design of earth retaining structures
Earth retaining structures are often used in geotechnical engineering and soil science to provide slope stability and support for infrastructure projects, such as roads, buildings, and bridges. These structures can be temporary or permanent, depending on the project requirements, and must be designed to resist the lateral pressure from the soil and water. The design of earth retaining structures involves considering various factors, such as soil properties, loading conditions, groundwater, and potential failure mechanisms.
There are several types of earth retaining structures, including gravity walls, cantilever walls, and sheet pile walls. Gravity walls rely on their own weight to resist the lateral forces acting on them and include massive concrete or stone structures. Cantilever walls use a combination of their weight and the retained soil's load to provide stability, featuring a vertical stem and a footing at the base. Sheet pile walls, on the other hand, consist of interconnected sheet piles driven into the ground to create a barrier that resists lateral pressure from the soil and water.
The design of earth retaining structures must also account for potential failure modes, such as sliding, overturning, and bearing capacity failure. Engineers use various analytical methods and numerical models to predict these failure mechanisms, ensuring the safe and efficient performance of the retaining structure. Proper design and selection of earth retaining structures play a crucial role in maintaining slope stability, protecting infrastructure, and reducing the risk of damage or collapse due to soil movement.
Construction and monitoring of slopes and retaining walls
The construction of slopes and retaining walls is a vital aspect of geotechnical engineering and soil science projects, requiring careful planning, execution, and quality control to ensure their stability and performance. Construction methods vary depending on the specific project requirements and site conditions, but may include excavation, compaction, backfilling, and the installation of drainage systems to manage groundwater.
Construction of earth retaining structures, such as gravity walls, cantilever walls, and sheet pile walls, also require specialized techniques and equipment. For example, sheet pile walls require the use of vibratory or impact hammers for driving the sheet piles into the ground. Proper construction practices are crucial in ensuring that the retaining structures perform as intended and provide adequate slope stability.
Monitoring the performance of slopes and retaining walls after construction is essential in detecting potential signs of instability and ensuring the structures' long-term safety. Monitoring activities may include visual inspections, instrumentation, and remote sensing techniques, such as LiDAR and satellite imagery. By continuously monitoring the condition of slopes and retaining walls, geotechnical engineers and soil scientists can identify and address issues before they escalate, protecting the surrounding environment and infrastructure from potential slope failure and damage.
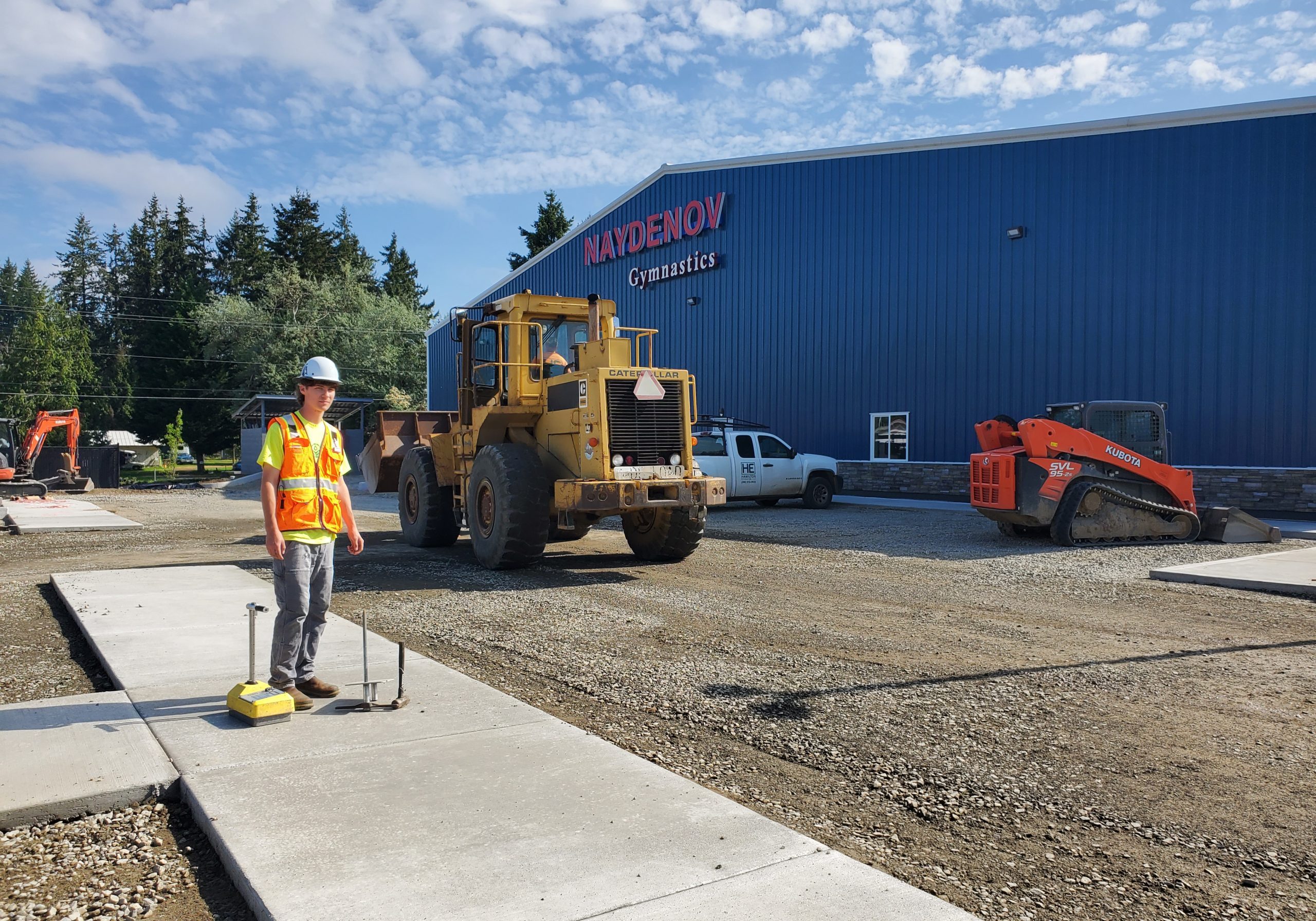
Ethics and Sustainability in Geotechnical Engineering and Soil Science
Environmental considerations
Environmental considerations play a significant role in geotechnical engineering and soil science as they directly affect the natural resources and ecosystems. Practitioners must be mindful of the potential impact their actions can have on the environment and take appropriate measures to minimize disturbance to natural habitats when carrying out site investigations and developing construction plans. This entails assessing the physical, biological, and chemical composition of soil and applying principles of ecological sustainability when managing and conserving soil resources.
It is essential for geotechnical engineers and soil scientists to be knowledgeable about environmental laws and regulations that are applicable to their projects. They must ensure that their work adheres to the guidelines set forth by various regulatory bodies to avoid penalties or legal ramifications. Moreover, understanding the potential impact of contaminants, pollutants, and invasive species on soil health and ecosystems is vital in preventing long-term environmental damage and ensuring sustainable engineering practices.
Collaboration with other interdisciplinary teams, such as ecologists, conservationists, and environmental scientists, is crucial in addressing environmental concerns and implementing sustainable solutions. By integrating a multidisciplinary approach, geotechnical engineers and soil scientists can contribute to ecologically-sensitive design and construction practices that minimize harm to the environment, promote biodiversity, and support environmental restoration efforts.
Sustainable construction practices
Sustainable construction practices in geotechnical engineering and soil science involve utilizing resource-efficient methods and materials to minimize environmental impact while maintaining safety and functionality. This includes selecting locally-sourced construction materials, employing measures to reduce energy consumption, and promoting the use of recyclable and renewable resources in the design and implementation of projects.
A significant aspect of sustainable construction is incorporating green infrastructure into the design and layout of projects. This involves integrating features like green roofs, rainwater harvesting systems, permeable pavements, and other low-impact development techniques to manage stormwater runoff, reduce heat island effect, and improve air quality. By implementing these practices, geotechnical engineers and soil scientists can contribute to sustainable urban development and reduce the overall environmental footprint of their projects.
Another element of sustainable construction is erosion and sediment control. Geotechnical engineers and soil scientists must design and implement preventative measures to minimize soil loss, protect adjacent water bodies, and prevent damage to the local ecosystem. This can be achieved by employing tools such as silt fences, sediment basins, and revegetation of disturbed areas. By emphasizing soil conservation and erosion control, professionals in this field can ensure the long-term ecological health of the construction site and contribute to the overall sustainability of their projects.
Ethical considerations in geotechnical engineering
Ethical considerations play a crucial role in the practice of geotechnical engineering and soil science, as professionals must ensure that their work is carried out with integrity, transparency, and social responsibility. One key aspect of professional ethics is the adherence to applicable codes of conduct, standards, and legislation in the design, investigation, and construction stages of projects. By following these guidelines, practitioners can ensure they are protecting public safety, health, and welfare while upholding industry best practices.
Geotechnical engineers and soil scientists must also bear the responsibility of ensuring that their actions do not unfairly disadvantage or burden certain social groups or communities. This includes the consideration of potential environmental justice issues and the equitable distribution of benefits and risks associated with their projects. By taking into account social and environmental impacts, professionals are better equipped to navigate ethical dilemmas and make decisions that promote public good and social equity.
Lastly, maintaining professional integrity and honest communication with clients, colleagues, and the public is paramount in upholding ethical standards in geotechnical engineering and soil science. Practitioners should not engage in fraudulent activities or provide misleading information, and they must remain transparent regarding potential conflicts of interest or limitations in their expertise. By doing so, they can foster trustworthiness and credibility within their profession and contribute ethically to the advancement of their field.
Geotechnical risk management
Geotechnical risk management is an essential aspect of geotechnical engineering and soil science, as it enables professionals to identify, assess, and mitigate potential hazards and uncertainties associated with their projects. This process involves conducting comprehensive site investigations, utilizing advanced analytical tools, and employing engineering judgment to develop a clear understanding of potential risks and their consequences.
Effective geotechnical risk management requires active communication and collaboration between various stakeholders, including clients, design teams, contractors, and regulatory agencies. By fostering open dialogue and sharing pertinent information, professionals are better able to manage uncertainties and make informed decisions in the design and construction processes. This collaborative approach not only helps mitigate potential risks but also contributes to more efficient project execution and cost-effective solutions.
Geotechnical risk management also necessitates ongoing monitoring and evaluation of project performance throughout its life cycle. This includes regular inspections, geotechnical instrumentation, and performance monitoring systems that provide real-time data to help identify potential issues and inform corrective actions. By implementing a robust risk management framework, geotechnical engineers and soil scientists can ensure that their projects are built safely, sustainably, and with minimal impact to the environment and surrounding communities.
We do what you need done
- NPDES Permitting, National Pollutant Discharge Elimination System (NPDES), EPA. What is NPDES? The NPDES permit program addresses water pollution by regulating point sources that discharge pollutants to waters of the United States.
- Turbidity Testing & PH Testing
- Erosion Compliance Inspection
- State & Federal Agency Correspondence
- Slope stability analyses
- Site reconnaissance
- Geotechnical engineering studies
- Retaining wall design
- Deep foundation design
- Micro pile design
- Helical earth anchor design
- Infiltration testing
- Soil science
- Soil laboratory testing
- Streambank restoration
- Compaction testing
- Special inspections
- Concrete testing
- ACI, American Concrete Institute
- Reinforced steel inspection
- ASTM, American standards testing methods.
- Construction Monitoring
- Earthwork construction
- QC, quality control
- QA, quality assurance
- Compaction testing
Trusted By Our Industry
We are in the field with you
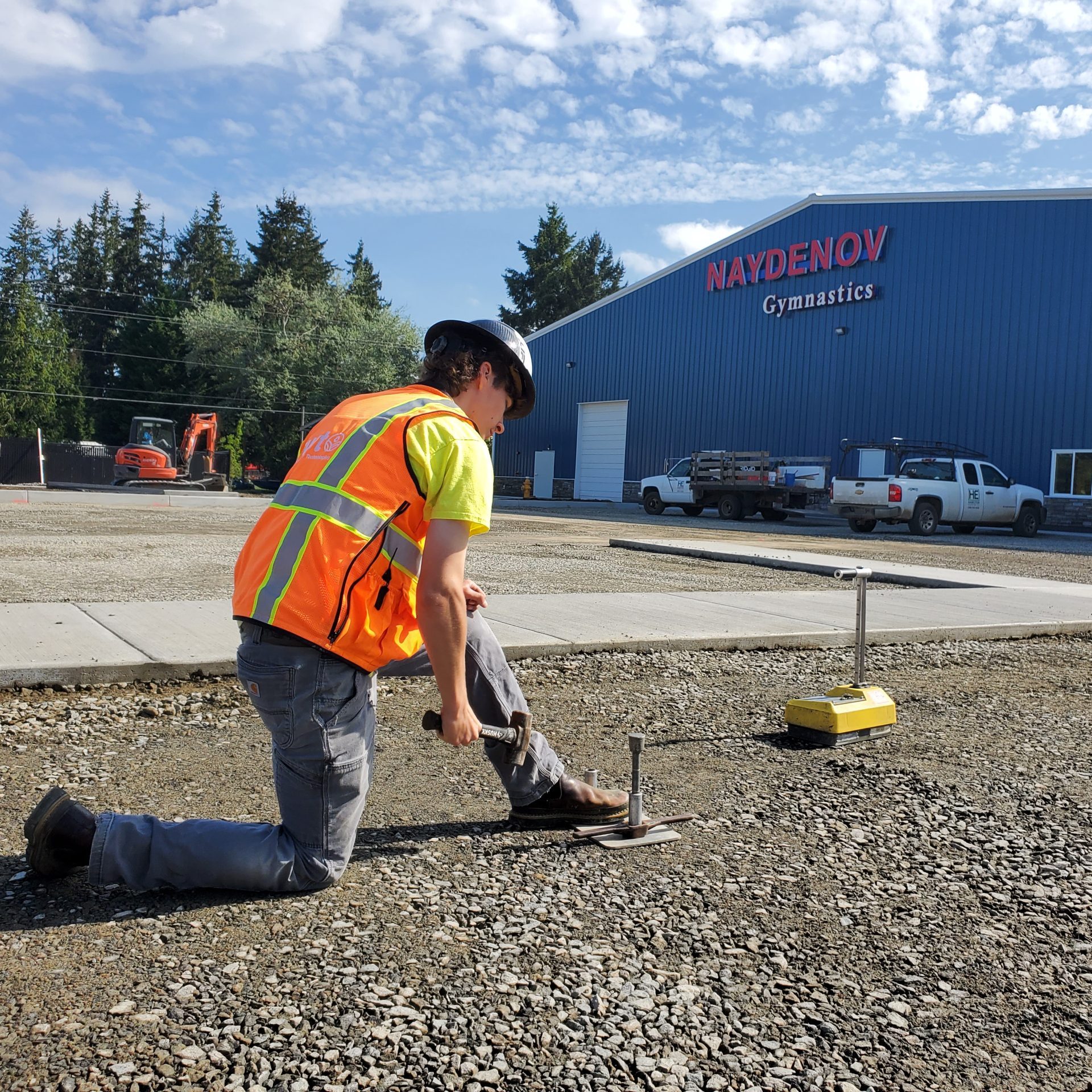
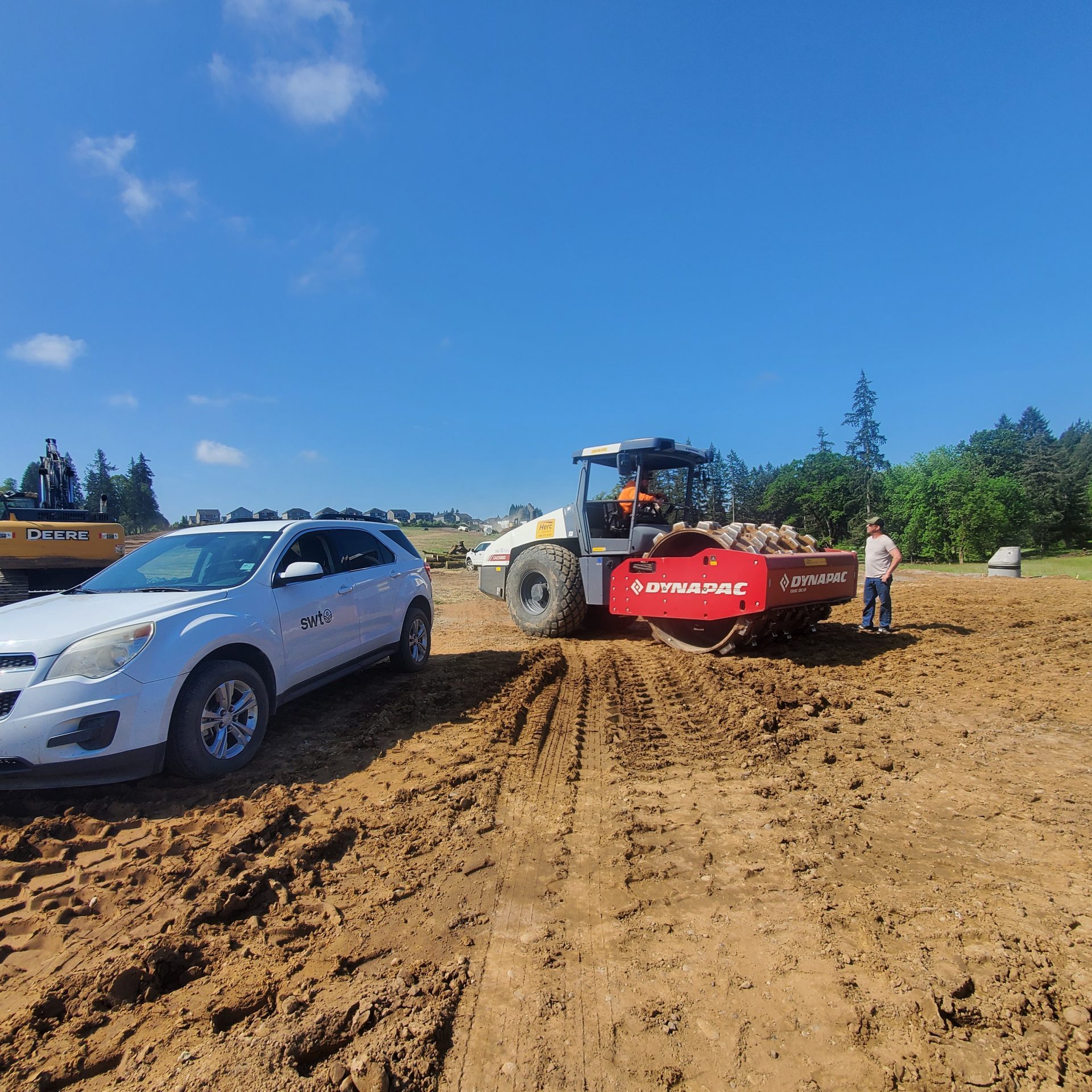
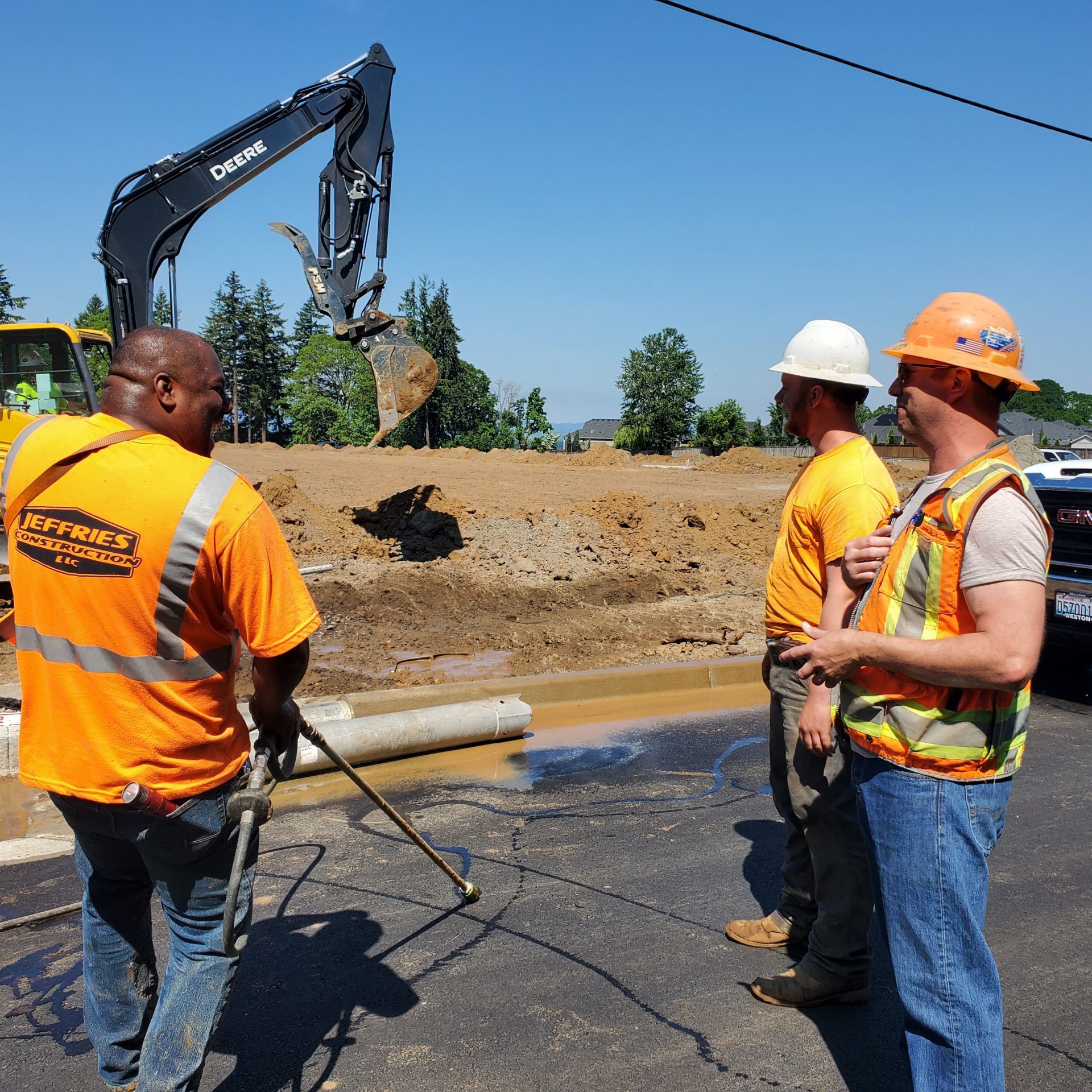
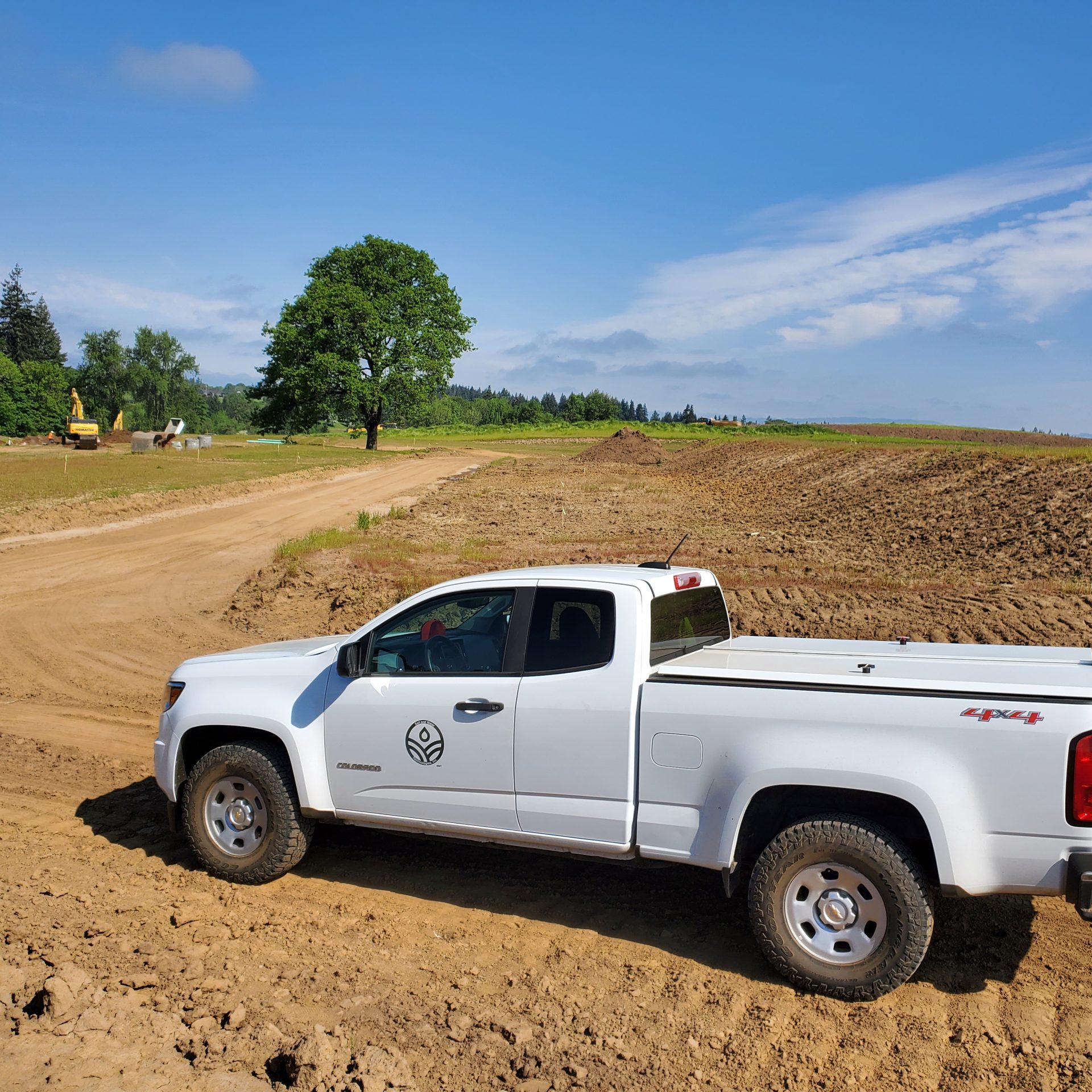
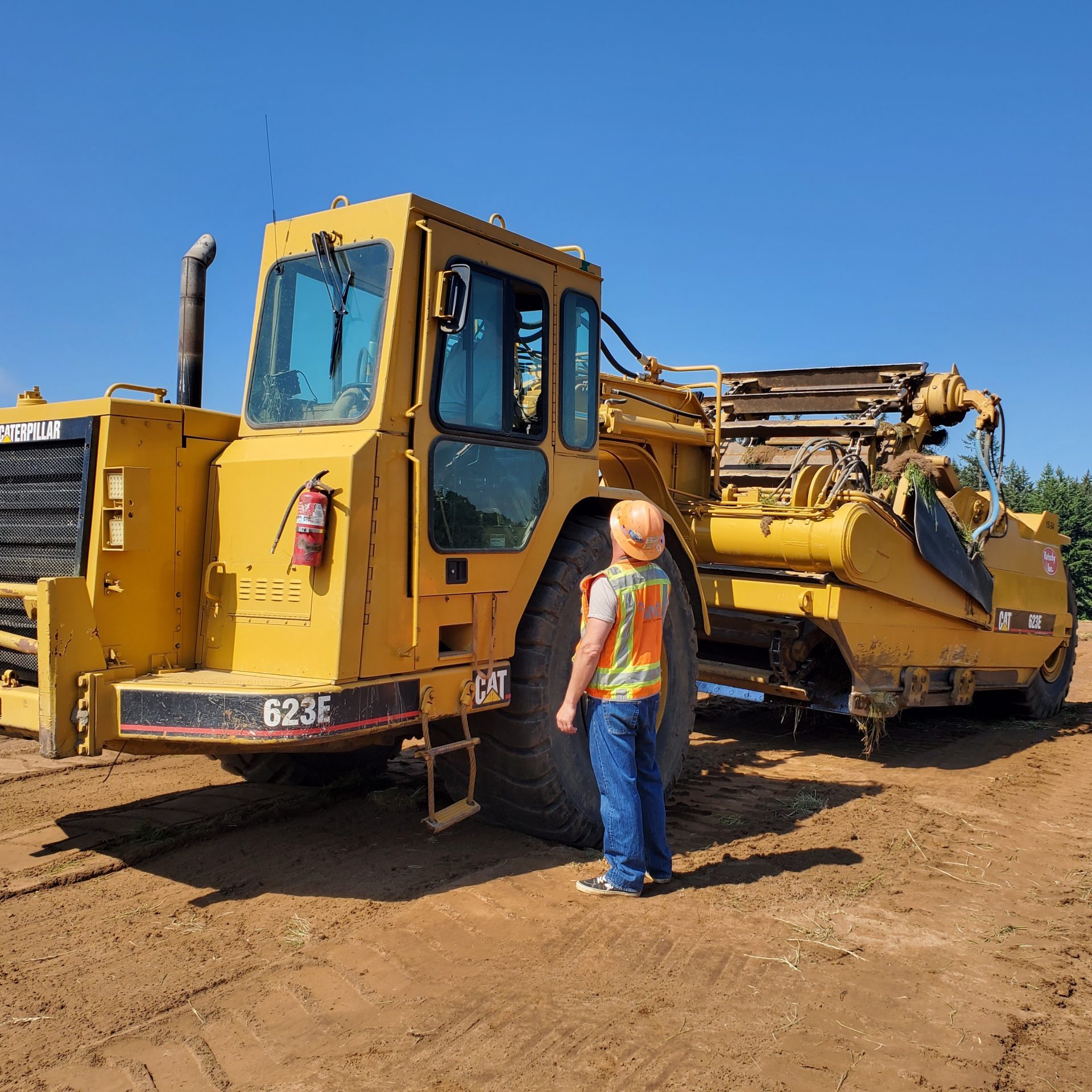